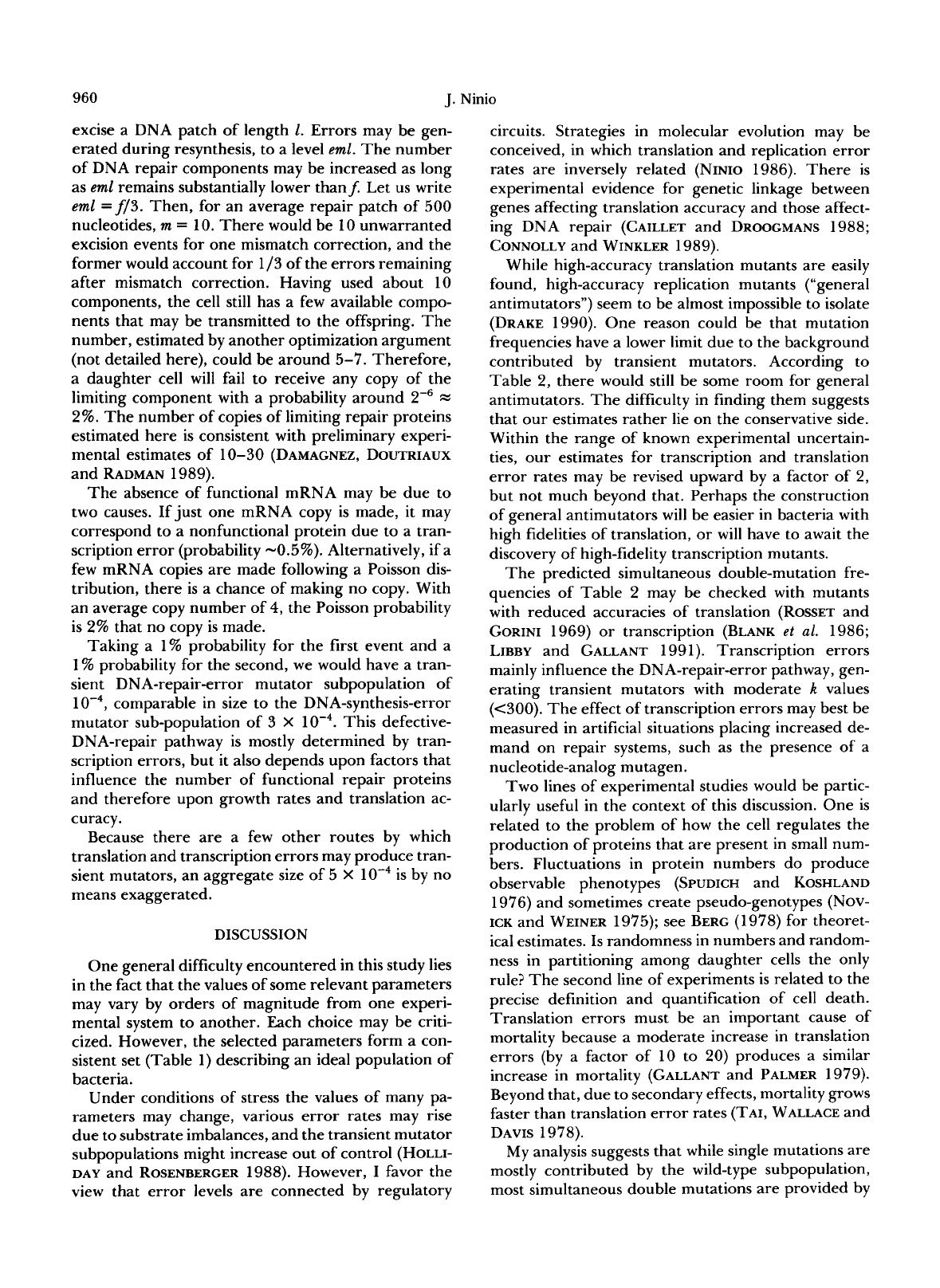
960
J.
Ninio
excise a DNA patch of length
1.
Errors may be gen-
erated during resynthesis, to a
level
eml.
The number
of DNA repair components may be increased as long
as
eml
remains substantially lower thanf. Let us write
em1
=
f/3. Then, for an average repair patch of 500
nucleotides,
m
=
10. There would be 10 unwarranted
excision events for one mismatch correction, and the
former would account for 1/3 of the errors remaining
after mismatch correction. Having used about 10
components, the cell still has a few available compo-
nents that may be transmitted to the offspring. The
number, estimated by another optimization argument
(not detailed here), could be around 5-7. Therefore,
a daughter cell
will
fail to receive any copy of the
limiting component with a probability around
2-6
=
2%.
The number of copies of limiting repair proteins
estimated here is consistent with preliminary experi-
mental estimates of 10-30 (DAMAGNEZ, DOUTRIAUX
and RADMAN 1989).
The absence of functional mRNA may
be
due to
two causes. If just one mRNA copy is made, it may
correspond to a nonfunctional protein due to a tran-
scription error (probability -0.5%). Alternatively, if a
few mRNA copies are made following a Poisson dis-
tribution, there is a chance of making no copy. With
an average copy number of
4,
the Poisson probability
is
2%
that no copy is made.
Taking a 1% probability for the first event and a
1%
probability for the second,
we
would have a tran-
sient DNA-repair-error mutator subpopulation of
comparable in size to the DNA-synthesis-error
mutator sub-population of 3
X
This defective-
DNA-repair pathway is mostly determined by tran-
scription errors, but it also depends upon factors that
influence the number of functional repair proteins
and therefore upon growth rates and translation ac-
curacy.
Because there are a few other routes by which
translation and transcription errors may produce tran-
sient mutators, an aggregate size of 5
x
is by no
means exaggerated.
DISCUSSION
One general difficulty encountered in this study lies
in the fact that the values of some relevant parameters
may vary by orders of magnitude from one experi-
mental system to another. Each choice may be criti-
cized. However, the selected parameters form a con-
sistent set (Table 1) describing an ideal population of
bacteria.
Under conditions of stress the values of many pa-
rameters may change, various
error
rates may rise
due to substrate imbalances, and the transient mutator
subpopulations might increase out of control (HOLLI-
DAY
and ROSENBERGER 1988). However,
I
favor the
view that error levels are connected
by
regulatory
circuits. Strategies in molecular evolution may be
conceived, in which translation and replication error
rates are inversely related (NINIO 1986). There is
experimental evidence for genetic linkage between
genes affecting translation accuracy and those affect-
ing DNA repair (CAILLET and DROOGMANS 1988;
CONNOLLY and WINKLER 1989).
While high-accuracy translation mutants are easily
found, high-accuracy replication mutants (“general
antimutators”) seem to be almost impossible to isolate
(DRAKE 1990). One reason could be that mutation
frequencies have a lower limit due to the background
contributed
by
transient mutators. According
to
Table
2,
there would still be some room for general
antimutators. The difficulty in finding them suggests
that our estimates rather lie on the conservative side.
Within the range of known experimental uncertain-
ties, our estimates for transcription and translation
error rates may
be
revised upward by a factor of
2,
but not much beyond that. Perhaps the construction
of general antimutators
will
be easier in bacteria with
high fidelities of translation, or
will
have to await the
discovery of high-fidelity transcription mutants.
The predicted simultaneous double-mutation fre-
quencies of Table
2
may be checked with mutants
with reduced accuracies of translation (ROSSET and
GORINI 1969) or transcription (BLANK
et
al.
1986;
LIBBY and GALLANT 1991). Transcription errors
mainly influence the DNA-repair-error pathway, gen-
erating transient mutators with moderate
K
values
(<300). The effect of transcription errors may best be
measured in artificial situations placing increased de-
mand on repair systems, such as the presence of a
nucleotide-analog mutagen.
Two lines of experimental studies would be partic-
ularly useful in the context of this discussion. One is
related to the problem of how the cell regulates the
production of proteins that are present in small num-
bers. Fluctuations in protein numbers do produce
observable phenotypes (SPUDICH and
KOSHLAND
1976) and sometimes create pseudo-genotypes (NOV-
ICK
and WEINER 1975); see BERG (1978) for theoret-
ical estimates.
Is
randomness in numbers and random-
ness in partitioning among daughter cells the only
rule? The second line of experiments is related to the
precise definition and quantification of cell death.
Translation errors must be an important cause
of
mortality because a moderate increase in translation
errors (by a factor of 10 to
20)
produces a similar
increase in mortality (GALLANT and PALMER 1979).
Beyond that, due to secondary effects, mortality grows
faster than translation error rates
(TAI,
WALLACE and
DAVIS 1978).
My
analysis suggests that while single mutations are
mostly contributed by the wild-type subpopulation,
most simultaneous double mutations are provided by